Pioneering Next-Gen Healthcare with AI, Epigenetics, and Bioengineering
Bioengineering stands at the precipice of a transformative era, where the convergence of biology, engineering, and technology promises to redefine the very fabric of human existence. This is not hyperbole. It’s a reflection of the rapid strides being...
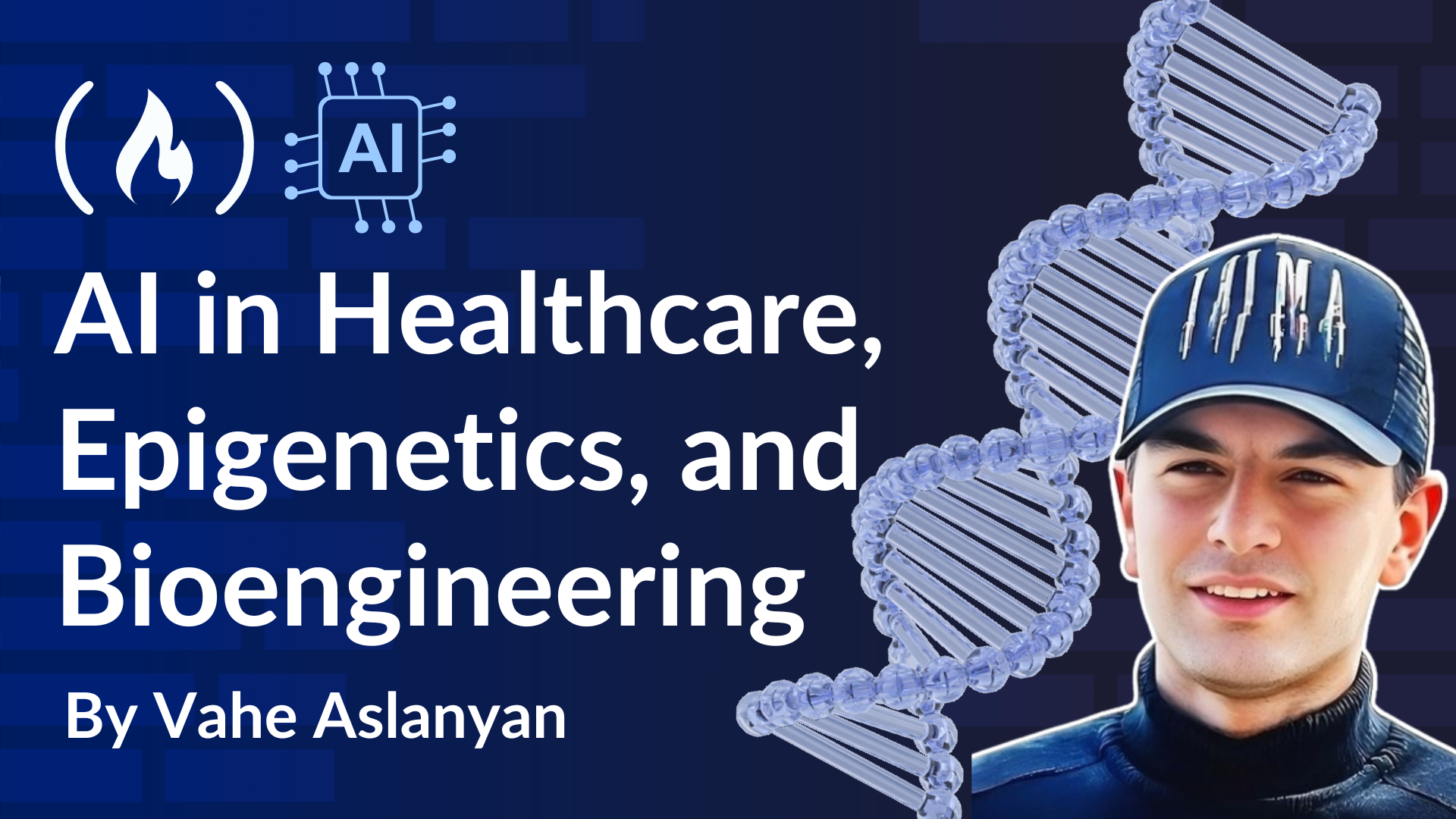
Bioengineering stands at the precipice of a transformative era, where the convergence of biology, engineering, and technology promises to redefine the very fabric of human existence.
This is not hyperbole. It’s a reflection of the rapid strides being made in fields such as regenerative medicine, synthetic biology, gene editing, and bioelectronics.
These advancements are not merely incremental improvements—they are paradigm shifts that challenge our understanding of biology and open doors to possibilities once confined to science fiction.
To fully appreciate the scope of this transformation, we need to delve into the intricate mechanics of these innovations and their profound implications for society..
If you want a comprehensive career guide that’ll help you get started in bioengineering, you can download this Bioengineering Playbook here.
And if you’d like to listen along as well, here’s the podcast on the handbook:
Table of Contents
Prerequisites
Bioengineering is an ever-evolving field that doesn’t require advanced math or engineering skills to get started. But you will need curiosity, problem-solving skills, and a willingness to learn.
While some coding knowledge can be beneficial, especially for data analysis and bioinformatics, what truly matters is critical thinking and an understanding of how biological systems interact with technology.
Whether your background is in biology, chemistry, or even computer science, experience in any of these areas—paired with a hands-on approach—can help you navigate this interdisciplinary field and contribute to groundbreaking advancements in medicine, biotechnology, and environmental sustainability
An Overview of Bioengineering
At its core, bioengineering is about harnessing the principles of biology to solve complex problems. Whether it is designing organs from scratch, editing genes to eradicate diseases, or engineering microorganisms to clean up environmental pollutants, bioengineering represents a fusion of creativity and precision.
It is a discipline that thrives on interdisciplinary collaboration, drawing from fields as diverse as computer science, material science, and ethics. This interconnectedness is not just a feature—it is a necessity. The challenges that bioengineering seeks to address are multifaceted, requiring solutions that are as complex and nuanced as the problems themselves.
Use Cases for Bioengineering
One of the most compelling aspects of bioengineering is its potential to revolutionize healthcare. Imagine a world where organ shortages are a thing of the past because we can bioprint organs tailored to individual patients. Picture a future where diseases like cystic fibrosis or sickle cell anemia are eradicated through precise genetic editing.
These are not distant dreams. They are tangible goals supported by groundbreaking research and technological innovation. The implications for human health are staggering—not just in terms of curing diseases but in fundamentally altering how we approach medicine.
But healthcare is only one facet of bioengineering's potential. The same principles that allow us to manipulate biological systems for medical purposes can be applied to agriculture and environmental sustainability.
Bioengineered crops resistant to droughts and pests could address food security challenges in an era of climate change. Genetically modified algae capable of capturing carbon dioxide more efficiently than natural processes could play a pivotal role in combating global warming. These applications highlight the versatility of bioengineering as a tool for addressing some of humanity's most pressing challenges.
Ethical Challenges
Yet, with great power comes great responsibility. The ability to manipulate life at its most fundamental level raises profound ethical questions. Where do we draw the line between curing diseases and enhancing human traits? How do we ensure that these technologies are accessible to all, rather than exacerbating existing inequalities?
These are real issues that must be addressed as bioengineering continues to evolve. Navigating these challenges requires a careful balance between innovation and regulation—a task that demands input from scientists, policymakers, ethicists, and the public.
AI and Bioengineering
The integration of artificial intelligence (AI) into bioengineering further amplifies its potential while introducing new complexities. AI-driven tools can analyze vast datasets to identify patterns and make predictions that would be impossible for humans alone.
This capability is invaluable in personalized medicine, where treatments can be tailored to an individual's genetic makeup with unprecedented precision. But it also raises concerns about data privacy and the ethical use of AI in decision-making processes. Ensuring that these technologies are used responsibly will be critical in maximizing their benefits while minimizing potential harms.
Perhaps one of the most exciting aspects of bioengineering is its ability to push the boundaries of what it means to be human. Brain-computer interfaces (BCIs) that restore mobility to paralyzed individuals or enhance cognitive functions represent just the tip of the iceberg. As these technologies become more sophisticated, they could blur the line between biology and technology, creating new possibilities for human enhancement. While this prospect is exhilarating, it also demands careful consideration of its societal implications.
A Glimpse into the Future
Bioengineering's impact extends beyond individual applications—it has the potential to reshape entire industries and economies. From sustainable manufacturing processes powered by synthetic biology to new forms of data storage using DNA-based systems, bioengineering is poised to drive innovation across multiple sectors.
This economic potential underscores the importance of investing in research and development while fostering an ecosystem that supports collaboration and knowledge sharing.
Bioengineering represents a frontier where science meets imagination—a field that holds the promise of solving some of humanity's greatest challenges while raising profound questions about our relationship with life itself.
As we stand on the cusp of this new era, it is imperative that we approach it with both optimism and caution. By embracing interdisciplinary collaboration, addressing ethical dilemmas head-on, and ensuring equitable access to these technologies, we can harness the full potential of bioengineering to create a future that benefits all of humanity.
The future of bioengineering is exciting. With advancements in regenerative medicine, synthetic biology, gene editing, and bioelectronics, humanity is poised to enter an era where the impossible becomes possible. This transformation is not speculative but rooted in the rapid progress we are witnessing across scientific disciplines.
To fully appreciate the depth of what lies ahead, we must explore these innovations in detail, understanding their mechanisms, potential applications, and profound implications for society. Each breakthrough not only addresses existing challenges but also opens new avenues for exploration and improvement. As we delve deeper, the interconnectedness of these fields becomes apparent, highlighting a synergistic approach to solving complex global issues.
Regenerative Medicine
Transformative Potential of Regenerative Medicine
Regenerative medicine has the potential to be one of the most transformative fields in bioengineering, offering solutions to some of humanity’s most pressing medical challenges: organ shortages, irreversible tissue damage, and chronic diseases.
At its core lies the revolutionary technology of 3D bioprinting, which uses bioinks composed of living cells to construct tissues and organs layer by layer. This technology is not just about creating structures; it is about engineering life itself, enabling the regeneration of complex biological systems that can integrate seamlessly with the human body.
Beyond bioprinting, advancements in stem cell research and tissue engineering are paving the way for creating personalized medical treatments tailored to individual genetic profiles. These innovations promise to revolutionize transplantation medicine, reduce dependency on donor organs, and eliminate the risks associated with immune rejection. The convergence of these technologies marks a significant leap towards achieving functional and sustainable regenerative therapies.
The Revolution of 3D Bioprinting
Imagine a world where patients with end-stage organ failure no longer wait anxiously for a donor match. Instead, their own cells are harvested, expanded, and used to print a new organ perfectly tailored to their body. This eliminates the risk of immune rejection and removes the need for lifelong immunosuppressive drugs, significantly improving patient outcomes and quality of life.
The implications are staggering: millions of lives saved, healthcare costs reduced, and a paradigm shift in how we view organ transplantation and medical treatment. Beyond this, the ability to produce organs on-demand could alleviate the strain on existing organ donation systems, making life-saving treatments more accessible to those in need. This vision of personalized organ generation not only enhances medical capabilities but also fosters a more equitable healthcare landscape.
Personalized Organ Generation and Its Implications
But 3D bioprinting is only the beginning. Researchers are now developing organoids, miniature lab-grown versions of human organs that mimic their structure and function. These organoids are invaluable for drug testing—eliminating the ethical concerns of animal testing—and serve as models to study complex diseases like Alzheimer’s or Parkinson’s at an unprecedented level of detail.
The ability to observe disease progression in real-time within a lab-grown brain or liver could unlock treatments that have eluded us for decades, accelerating the pace of medical discoveries and therapeutic interventions.
Organoids also provide a platform for personalized medicine, allowing for the testing of drug responses on a person’s specific cellular makeup. This not only enhances the precision of treatments but also reduces the time and cost associated with bringing new drugs to market.
Development and Further Applications of Organoids
Regenerative medicine also extends into treating chronic conditions, offering innovative solutions that go beyond symptom management. For example, bioengineered pancreatic islets could restore insulin production in diabetic patients, addressing the root cause of the disease rather than merely controlling blood sugar levels.
Similarly, cardiac patches made from a patient’s own cells could repair damaged heart tissue after a heart attack, significantly improving recovery outcomes and reducing the likelihood of subsequent cardiac events.
These innovations promise not only to save lives but also to enhance the quality of life for people suffering from chronic ailments, providing long-term solutions that were previously unattainable.
By targeting the fundamental biological mechanisms underlying these conditions, regenerative medicine offers a pathway to sustainable health improvements and reduced dependence on lifelong medical interventions.
Regenerative Solutions for Chronic Conditions
Still, the challenges remain significant as the field progresses towards widespread application. Scaling up bioprinting for mass production while maintaining precision and affordability is no small feat, requiring advancements in both technology and manufacturing processes.
Also, ethical questions surrounding access to these technologies must be addressed to ensure equitable distribution, preventing disparities in who can benefit from these medical breakthroughs.
Regulatory frameworks need to evolve in tandem with technological advancements to ensure safety, efficacy, and ethical compliance, fostering public trust and acceptance. Also, the integration of regenerative therapies into existing healthcare systems necessitates comprehensive training for medical professionals and the development of new infrastructure to support these advanced treatments.
Addressing these challenges is crucial for realizing the full potential of regenerative medicine and ensuring that its benefits are accessible to all segments of society.
Gene Editing
Gene Editing Technologies and Their Scope
Gene editing technologies like CRISPR-Cas9 have ushered in an era where we can manipulate the very blueprint of life with surgical precision.
While much attention has been given to curing genetic disorders such as cystic fibrosis or sickle cell anemia, the true potential of gene editing lies in its ability to create entirely new biological paradigms. This transformative capability extends beyond disease treatment, encompassing the enhancement of human traits, agricultural improvements, and environmental conservation efforts.
The precision and versatility of gene editing tools enable scientists to make targeted modifications at the DNA level, opening up possibilities that were once confined to the realm of science fiction.
As these technologies continue to advance, they offer unprecedented control over genetic material, allowing for the customization and optimization of living organisms for specific purposes. The ethical and societal implications of such profound control over life’s genetic code necessitate careful consideration and responsible stewardship.
Gene Drives and Disease Eradication
One groundbreaking concept is gene drives, which could be used to eradicate vector-borne diseases like malaria by altering mosquito populations so they can no longer transmit the parasite.
This approach has already shown promise in controlled environments and could save hundreds of thousands of lives annually if implemented responsibly. By ensuring that the modified genes spread rapidly through mosquito populations, gene drives offer a self-sustaining solution to reduce disease transmission without the need for continuous intervention.
But the deployment of gene drives must be carefully managed to prevent unintended ecological consequences, such as disrupting food chains or affecting non-target species. Collaborative efforts between scientists, policymakers, and communities are essential to ensure that gene drive technologies are used ethically and effectively, balancing the benefits of disease eradication with the preservation of ecological integrity.
The successful application of gene drives could revolutionize public health strategies, providing a powerful tool in the fight against some of the world's most devastating diseases.
Synthetic Biology's Role in Environmental Sustainability
Beyond disease eradication, gene editing paves the way for synthetic biology, where scientists design organisms with entirely new functions.
Imagine bacteria engineered to consume plastic waste in oceans or plants modified to sequester carbon dioxide at rates far beyond natural photosynthesis. These innovations could address some of our most pressing environmental challenges while simultaneously creating new industries centered around sustainable biotechnology.
Engineered organisms can be tailored to perform specific tasks, such as breaking down pollutants, producing biofuels, or enhancing crop resilience, offering versatile solutions to complex problems.
The integration of gene editing with synthetic biology enhances our ability to innovate, enabling the creation of biological systems that complement and enhance natural processes. As these technologies mature, they hold the promise of transforming industries, improving environmental sustainability, and fostering a more resilient and adaptable global ecosystem.
Ethical Considerations in Gene Editing
We must keep in mind, though, that gene editing also raises profound ethical questions that society must address as these technologies become more accessible and powerful. Where do we draw the line between curing diseases and enhancing human traits? Should we allow genetic modifications that improve intelligence or physical abilities, potentially leading to a new era of human enhancement?
These are not hypothetical scenarios but imminent dilemmas that society must grapple with as the technology matures.
The potential for misuse or unintended consequences necessitates robust ethical guidelines and regulatory frameworks to ensure responsible use of gene editing technologies. Public discourse and inclusive decision-making processes are crucial to navigate the moral landscape of genetic manipulation, balancing the pursuit of scientific advancement with the preservation of ethical standards and societal values.
Addressing these ethical challenges is essential for harnessing the benefits of gene editing while mitigating risks and ensuring that its applications align with the broader interests of humanity.
Advancements in Epigenetic Editing
The future may also see advancements in epigenetic editing—modifying how genes are expressed without altering their underlying DNA sequence. This could allow scientists to "turn off" harmful genes or "activate" beneficial ones dynamically, offering new ways to treat diseases like cancer or autoimmune disorders.
Unlike traditional gene editing, which involves permanent changes to the genetic code, epigenetic modifications are reversible and can be tailored to respond to environmental factors or therapeutic needs.
This flexibility provides a more nuanced approach to genetic intervention, enabling precise control over gene expression and reducing the risk of unintended genetic alterations. Epigenetic editing holds promise for personalized medicine, allowing treatments to be customized based on an individual’s unique genetic and epigenetic profile. By targeting the regulatory mechanisms that control gene activity, scientists can develop sophisticated therapies that address the root causes of diseases while minimizing side effects and enhancing overall treatment efficacy.
Personalized Medicine
Personalized Medicine's Integration with AI
The integration of genomics, proteomics, and artificial intelligence (AI) is transforming medicine into a highly personalized discipline.
Unlike traditional approaches that treat patients based on generalized protocols, personalized medicine tailors interventions to an individual’s unique genetic makeup and lifestyle factors. This shift towards customization enhances the precision and effectiveness of medical treatments, reducing the trial-and-error approach that has long characterized healthcare.
By leveraging vast amounts of biological and clinical data, personalized medicine enables the identification of specific biomarkers and genetic variations that influence disease risk, treatment response, and overall health outcomes.
This data-driven approach not only improves patient care but also fosters a deeper understanding of the complex interactions between genetics, environment, and behavior. As personalized medicine continues to evolve, it promises to revolutionize healthcare delivery, making it more proactive, preventive, and patient-centric.
Targeted Cancer Therapies in Personalized Medicine
One area where this approach shines is oncology. By sequencing a tumor’s genome, oncologists can identify specific mutations driving cancer growth and select therapies that target those mutations directly. This not only improves efficacy but also minimizes debilitating side effects associated with conventional treatments like chemotherapy.
Targeted therapies, such as tyrosine kinase inhibitors or monoclonal antibodies, are designed to interfere with specific molecular pathways that cancer cells rely on for survival and proliferation.
By precisely attacking the mechanisms that enable cancer cells to thrive, these treatments can effectively shrink tumors and prolong patient survival with fewer adverse effects compared to traditional chemotherapy.
Also, the ability to monitor genetic changes in tumors over time allows for the adaptation of treatment plans in response to evolving cancer dynamics, enhancing the overall effectiveness of cancer care. Personalized oncology represents a paradigm shift in cancer treatment, moving from a one-size-fits-all approach to highly individualized therapeutic strategies.
Pharmacogenomics and Drug Metabolism
But personalized medicine goes beyond cancer care, extending its benefits to a wide range of medical disciplines. The emerging field of pharmacogenomics studies how genetic variations influence drug metabolism, efficacy, and toxicity. For instance, some individuals metabolize certain painkillers too quickly, rendering them ineffective, while others metabolize them too slowly, increasing the risk of overdose.
Understanding these variations allows physicians to prescribe medications—and dosages—optimized for each patient’s genetic profile, enhancing therapeutic outcomes and minimizing adverse reactions. This level of customization not only improves patient safety but also increases the likelihood of successful treatment, reducing the need for multiple medication trials and adjustments.
As pharmacogenomic testing becomes more accessible and integrated into routine healthcare, it holds the potential to significantly enhance the precision and personalization of medical treatments across various conditions.
Wearable Biosensors and Real-Time Health Monitoring
Wearable biosensors further enhance personalization by enabling real-time health monitoring. Devices capable of analyzing biomarkers such as glucose levels or cardiac rhythms continuously provide actionable insights that empower patients to take proactive steps in managing their health. These sensors collect vast amounts of data, which, when analyzed by AI algorithms, can predict disease risks based on historical and real-time information.
For example, wearable devices can detect irregular heartbeats that may indicate atrial fibrillation, prompting early medical intervention to prevent strokes. Similarly, continuous glucose monitors help diabetic patients maintain optimal blood sugar levels, reducing the risk of complications. The integration of wearable biosensors into daily life transforms healthcare from a reactive to a preventive model, allowing for timely interventions and more effective management of chronic conditions.
Ensuring Equitable Access to Personalized Medicine
The challenge lies in democratizing access to personalized medicine technologies to ensure that their benefits are equitably distributed. Without equitable distribution mechanisms, there is a risk that these advancements will widen existing healthcare disparities rather than bridge them.
Factors such as socioeconomic status, geographic location, and access to healthcare infrastructure can influence who benefits from personalized medicine, potentially exacerbating inequalities in health outcomes.
To address this, policies and initiatives must focus on making personalized medicine accessible and affordable for all populations, regardless of their background or circumstances. This includes investing in healthcare infrastructure, providing education and training for healthcare professionals, and implementing regulatory frameworks that support the widespread adoption of personalized therapies.
Ensuring equitable access to personalized medicine is essential for maximizing its societal benefits and fostering a more inclusive and effective healthcare system.
Bioelectronic Medicine
Bioelectronic Medicine's Innovations
Bioelectronic medicine represents a frontier where biology meets electronics to create devices that interface directly with the nervous system. These innovations have profound implications for treating chronic diseases and even enhancing human capabilities, offering a new dimension of therapeutic interventions that go beyond traditional pharmacological approaches.
By leveraging the intricate communication pathways of the nervous system, bioelectronic devices can modulate neural activity with high precision, providing targeted treatments for a variety of conditions.
This integration of electronics with biological systems enables real-time monitoring and intervention, enhancing the responsiveness and effectiveness of medical treatments. As bioelectronic technologies continue to advance, they hold the potential to revolutionize healthcare by offering minimally invasive, highly customizable, and efficient solutions for managing complex health issues.
The convergence of biology and electronics in medicine marks a significant step towards more integrated and intelligent healthcare systems.
Neural Prosthetics and Brain-Computer Interfaces
One groundbreaking application of bioelectronic medicine is in neural prosthetics—devices that restore mobility or sensory functions lost due to injury or disease. Advanced brain-computer interfaces (BCIs) now enable paralyzed individuals to control robotic limbs using only their thoughts—a feat once relegated to science fiction. These interfaces translate neural signals into commands that can operate external devices, providing individuals with restored functionality and independence.
As BCI technology continues to evolve, it may allow for more seamless integration between human cognition and external devices, effectively augmenting natural abilities and enhancing quality of life.
Beyond prosthetics, BCIs have potential applications in cognitive enhancement, enabling individuals to interact with digital systems more intuitively and efficiently. The development of more sophisticated and user-friendly neural interfaces could lead to breakthroughs in how humans interact with technology, blurring the lines between biological and artificial systems.
Bioelectronics in Treating Neurological Conditions
Bioelectronics also hold promise for treating conditions like epilepsy or depression through targeted nerve stimulation rather than pharmaceuticals. By delivering precise electrical impulses to specific neural circuits, these devices can modulate brain activity without the side effects associated with drugs. For instance, deep brain stimulation (DBS) has been successfully used to reduce seizure frequency in epilepsy patients and alleviate symptoms of major depressive disorder.
These non-invasive or minimally invasive treatments offer alternatives for people who do not respond well to traditional medications, providing more effective and personalized therapeutic options. Also, bioelectronic devices can be programmed to respond dynamically to physiological changes, adjusting stimulation parameters in real-time to optimize treatment efficacy. This adaptability enhances the precision and effectiveness of bioelectronic therapies, making them a valuable tool in the management of chronic neurological and psychiatric conditions.
Future of Implantable Bioelectronic Systems
Looking further ahead, researchers envision implantable bioelectronic systems capable of real-time health monitoring and intervention.
For instance, a pacemaker-like device could detect early signs of a heart attack and administer corrective electrical stimulation autonomously—a true lifesaver in critical situations. These systems could integrate multiple sensors to monitor various physiological parameters, providing comprehensive health data that can be used for continuous assessment and timely intervention.
The ability to autonomously respond to health emergencies could significantly reduce mortality rates and improve patient outcomes, particularly in acute medical situations where immediate action is crucial. Beyond this, the miniaturization and biocompatibility of bioelectronic devices are advancing, making them more comfortable and less invasive for patients.
The development of such systems represents a significant leap towards more intelligent and responsive healthcare solutions, where medical devices actively participate in maintaining and restoring health.
Synthetic Biology
Synthetic Biology's Diverse Applications
Synthetic biology takes bioengineering to its logical extreme by designing entirely new biological systems from scratch—or reprogramming existing ones for novel purposes. This field blurs the line between biology and engineering, creating organisms that perform tasks nature never intended and offering solutions to some of the most complex challenges facing humanity.
By combining principles from biology, engineering, computer science, and chemistry, synthetic biology enables the creation of custom-designed organisms with specific functionalities tailored to meet diverse needs.
This multidisciplinary approach fosters innovation and creativity, allowing scientists to rethink and redesign biological systems with unprecedented precision and versatility. As synthetic biology continues to evolve, it promises to revolutionize industries ranging from healthcare and agriculture to environmental management and manufacturing.
The ability to engineer life at the genetic level opens up a myriad of possibilities for improving human life and preserving the planet, making synthetic biology a cornerstone of future technological advancements.
Sustainable Manufacturing through Synthetic Biology
One compelling application of synthetic biology is in sustainable manufacturing. Engineered microbes can produce biofuels or biodegradable plastics from renewable resources like agricultural waste, reducing our reliance on fossil fuels and mitigating environmental pollution.
These microorganisms are designed to efficiently convert biomass into valuable products, offering a sustainable alternative to traditional manufacturing processes that are often resource-intensive and environmentally damaging.
The production of biodegradable plastics addresses the growing issue of plastic pollution, providing materials that break down naturally without harming ecosystems. By leveraging the metabolic pathways of engineered microbes, synthetic biology enables the creation of eco-friendly products that support a circular economy and promote environmental sustainability.
This approach not only reduces the carbon footprint of manufacturing but also fosters the development of green technologies that are essential for combating climate change and preserving natural resources.
Yeast Strains for Pharmaceutical Production
Similarly, yeast strains modified to synthesize pharmaceuticals could democratize access to life-saving drugs by enabling local production in resource-limited settings.
Traditional pharmaceutical manufacturing often involves complex and expensive processes that limit the availability of essential medications in developing regions. Engineered yeast can produce a wide range of drugs more efficiently and cost-effectively, making treatments more accessible to underserved populations.
This approach also enhances the scalability and flexibility of drug production, allowing for rapid responses to emerging health crises and the customization of medications to meet specific therapeutic needs.
By decentralizing pharmaceutical production, synthetic biology can play a pivotal role in improving global health outcomes and ensuring that life-saving treatments are available to all, regardless of geographic or economic barriers. The ability to produce pharmaceuticals locally not only increases accessibility but also reduces dependency on global supply chains, enhancing the resilience of healthcare systems worldwide.
Biological Computing and Data Storage
Another visionary concept within synthetic biology is biological computing, where living cells are programmed to perform computational tasks traditionally handled by silicon-based systems. While still in its infancy, this approach could revolutionize data storage and processing by leveraging DNA’s unparalleled density and efficiency.
One gram of DNA can theoretically store 215 petabytes of information, offering a solution to the growing demand for data storage in our increasingly digital world.
Biological computing harnesses the natural properties of living organisms to perform complex computations, enabling the development of highly efficient and scalable computing systems.
This innovative approach not only addresses the limitations of traditional computing technologies but also opens up new possibilities for bio-integrated devices and intelligent systems. As research progresses, biological computing could lead to breakthroughs in fields such as artificial intelligence, biotechnology, and nanotechnology, driving the next wave of technological innovation.
Synthetic Biology in Resilient Agriculture
Synthetic biology also has potential applications in agriculture through crops engineered for resilience against droughts or pests without relying on chemical pesticides or fertilizers.
These innovations could enhance food security while reducing ecological harm, creating a win-win scenario for both humanity and the planet. Genetically modified crops can be designed to thrive in harsh environmental conditions, ensuring stable yields even in the face of climate change and resource scarcity.
Pest-resistant crops also reduce the need for chemical interventions, minimizing the environmental impact of agriculture and promoting sustainable farming practices. By improving the resilience and sustainability of food production, synthetic biology contributes to the resilience and productivity of agricultural systems, addressing the dual challenges of feeding a growing population and protecting the environment.
These advancements not only support agricultural productivity but also align with broader environmental and sustainability goals, demonstrating the multifaceted benefits of synthetic biology in addressing some of the most critical challenges of our time.
Environmental Bioengineering
Environmental Bioengineering's Impact
The same tools transforming healthcare can be applied to address environmental crises threatening our planet’s future. Bioengineers are developing solutions that leverage biology’s inherent adaptability and efficiency to tackle issues such as climate change and pollution, offering innovative approaches that complement traditional environmental management strategies.
By harnessing the power of living organisms and biological processes, environmental bioengineering provides sustainable and effective solutions to some of the most pressing environmental challenges.
These bioengineered systems can restore ecosystems, reduce greenhouse gas emissions, and remediate contaminated environments, contributing to the overall health and resilience of the planet.
As the urgency of addressing environmental degradation and climate change intensifies, the role of bioengineering in environmental conservation and sustainability becomes increasingly vital. The integration of biological innovations with environmental stewardship represents a holistic approach to safeguarding the planet for future generations.
Genetically Modified Algae for Carbon Capture
For example, genetically modified algae with enhanced photosynthetic efficiency could capture carbon dioxide from industrial emissions more effectively than current technologies allow. These algae can convert captured carbon into biomass, which can then be processed into biofuels or other valuable products, effectively turning waste into wealth while mitigating global warming.
Enhanced photosynthesis in algae not only reduces the concentration of greenhouse gases in the atmosphere but also provides a renewable source of energy that can replace fossil fuels.
Also, algae-based carbon capture systems are scalable and can be integrated into existing industrial infrastructure, offering a practical solution for reducing carbon footprints on a large scale. The dual benefits of carbon sequestration and biofuel production make genetically modified algae a promising tool in the fight against climate change, contributing to both environmental sustainability and energy security.
Biodegradable Plastics as Pollution Solutions
Similarly, bioengineered materials designed to degrade naturally offer an elegant solution to plastic pollution choking ecosystems worldwide.
Unlike traditional plastics derived from petrochemicals, these materials break down into harmless components when exposed to environmental conditions like sunlight or moisture, reducing the long-term impact of plastic waste on wildlife and natural habitats. Biodegradable plastics can be engineered to maintain their durability and functionality during use while ensuring that they decompose safely after disposal, addressing the environmental hazards associated with conventional plastics.
Furthermore, the development of bio-based plastics supports the transition towards a circular economy, where materials are continuously reused and recycled, minimizing waste and conserving natural resources.
By providing sustainable alternatives to traditional plastics, bioengineered materials play a crucial role in reducing pollution and promoting environmental health, aligning with global efforts to protect and preserve ecosystems.
Bioengineered Crops for Climate Resilience
In agriculture, bioengineered crops resistant to extreme weather conditions promise greater yields amid climate instability—a critical development as global populations rise and arable land diminishes.
These crops can be designed to withstand droughts, floods, and temperature extremes, ensuring stable food production even in the face of unpredictable climate patterns. Enhanced resilience in crops not only supports food security but also reduces the need for resource-intensive interventions, such as irrigation and artificial heating, promoting more sustainable agricultural practices.
Bioengineered crops can also be tailored to utilize nutrients more efficiently, reducing the environmental impact of farming by minimizing fertilizer runoff and soil degradation.
By improving the adaptability and sustainability of agricultural systems, bioengineered crops contribute to the resilience and productivity of food production, addressing the dual challenges of feeding a growing population and protecting the environment.
Aging and Longevity
Longevity Science and Human Lifespan
Advances in bioengineering hold tantalizing prospects for extending human lifespan while improving quality of life during aging—a field often referred to as “longevity science.”
By targeting the biological mechanisms that drive aging and age-related diseases, bioengineers aim to slow down or even reverse the aging process, enabling individuals to live longer, healthier lives. This ambitious goal involves a multidisciplinary approach, integrating insights from genetics, molecular biology, regenerative medicine, and bioinformatics to develop comprehensive strategies for promoting longevity.
The potential to extend healthy lifespan not only has profound implications for individual well-being but also for societal structures, including healthcare systems, economies, and social support networks. As research in longevity science progresses, it promises to unlock new understanding of the aging process and pave the way for innovative interventions that enhance human health and longevity.
Targeting Cellular Senescence for Anti-Aging
One promising avenue involves targeting cellular senescence—the process by which cells lose their ability to divide but remain metabolically active, contributing to inflammation and tissue dysfunction over time.
By removing senescent cells or reversing their effects through small molecules known as senolytics, researchers aim to delay age-related diseases like arthritis or Alzheimer’s significantly. Senolytic therapies have shown promise in preclinical studies, demonstrating the ability to improve physical function and extend lifespan in animal models.
By clearing senescent cells, these treatments reduce chronic inflammation and tissue damage, addressing some of the root causes of aging and promoting healthier aging processes. As senolytic therapies advance through clinical trials, they hold the potential to become a cornerstone of anti-aging medicine, offering new ways to enhance healthspan—the period of life spent in good health—alongside lifespan.
Enhancing DNA Repair Mechanisms
Another strategy focuses on enhancing DNA repair mechanisms compromised over time due to accumulated damage from environmental stressors like toxins or radiation exposure.
As we age, the accumulation of DNA damage can lead to cellular dysfunction, mutations, and the development of age-related diseases. Bioengineering approaches aim to bolster the body's natural DNA repair processes, ensuring that cells maintain their integrity and functionality throughout life.
Techniques such as gene therapy and CRISPR-based gene editing are being explored to enhance the expression of DNA repair enzymes or correct genetic mutations that impair repair mechanisms. By improving the efficiency and accuracy of DNA repair, these interventions could slow down the aging process at the cellular level, reducing the incidence of diseases associated with genetic damage and promoting overall cellular health.
Enhanced DNA repair mechanisms not only contribute to longevity but also improve the resilience of cells against various forms of stress, supporting healthier and more robust aging.
Epigenetic Reprogramming and Rejuvenation
Epigenetic reprogramming offers another avenue by resetting biological clocks within cells—a process already demonstrated in animal models with promising results for rejuvenation therapies. Epigenetic modifications, which influence gene expression without altering the underlying DNA sequence, play a crucial role in regulating cellular function and aging.
By reprogramming the epigenetic state of cells, scientists can potentially reverse age-related changes, restoring youthful function and vitality.
This approach has shown success in extending the lifespan and improving healthspan in animal studies, indicating its potential applicability to humans. Epigenetic reprogramming could lead to therapies that not only extend lifespan but also enhance the quality of life during aging by maintaining cellular and tissue health.
As research progresses, epigenetic interventions may become a key component of anti-aging strategies, offering a way to rejuvenate cells and tissues and promote healthier, longer lives.
Ethical Challenges in Bioengineering
While these advancements promise immense benefits, they also raise significant ethical questions about equity, privacy, and safety risks from unintended consequences, such as ecological disruptions.
The rapid pace of bioengineering innovations often outstrips the development of regulatory and ethical frameworks necessary to guide their responsible use. Issues such as genetic privacy, consent for genetic modifications, and the potential for creating socioeconomic disparities through unequal access to advanced therapies require careful consideration.
The manipulation of biological systems also poses risks of unintended ecological impacts, particularly with technologies like gene drives and synthetic organisms that could disrupt existing ecosystems if not properly controlled. Addressing these ethical challenges is crucial to ensure that bioengineering technologies are developed and deployed in ways that are safe, equitable, and aligned with societal values.
The responsible stewardship of bioengineering advancements involves fostering transparent dialogue, establishing robust regulatory mechanisms, and promoting inclusive decision-making processes that consider the diverse perspectives and needs of all stakeholders.
Regulatory Frameworks for Bioengineering
Regulatory oversight frameworks must evolve in tandem with technological advancements to ensure public trustworthiness and global alignment of policies.
As bioengineering technologies become more sophisticated and widespread, consistent and comprehensive regulations are essential to govern their development, application, and distribution. International collaboration is also necessary to create standardized guidelines that address cross-border ethical and safety concerns, preventing regulatory loopholes and ensuring that bioengineering practices adhere to universal ethical principles.
These frameworks should encompass not only the approval and monitoring of new technologies but also mechanisms for ongoing assessment of their long-term impacts on society and the environment.
By establishing clear and consistent regulatory standards, policymakers can facilitate the responsible advancement of bioengineering while safeguarding public health and ethical integrity. It’s also crucial that regulatory bodies engage with scientific communities, industry stakeholders, and the public to stay informed about emerging technologies and to adapt regulations as needed, fostering a dynamic and responsive governance structure.
Fair Access and Affordability in Bioengineering
Ensuring fair access and affordability of bioengineering technologies is another critical ethical consideration. Without equitable distribution mechanisms, there is a risk that these advancements will widen existing healthcare disparities rather than bridge them.
High costs associated with cutting-edge therapies and technologies can limit access to wealthy individuals or regions, exacerbating inequalities in health outcomes and quality of life. To mitigate this, policies must focus on making bioengineering innovations accessible and affordable for all populations, regardless of their socioeconomic status or geographic location.
This includes investing in healthcare infrastructure, providing subsidies or insurance coverage for advanced treatments, and promoting global collaborations to distribute technologies equitably.
Efforts should also be made to support research and development in underserved areas, ensuring that the benefits of bioengineering advancements are shared broadly and do not disproportionately favor certain groups over others. By prioritizing equity in the distribution of bioengineering technologies, society can maximize their positive impact and promote a more just and inclusive healthcare landscape.
Societal Acceptance and Cultural Considerations
Societal acceptance and cultural considerations also play a significant role in the ethical deployment of bioengineering technologies.
Public perception and acceptance of bioengineering innovations can influence their adoption and success, making it essential to engage communities in discussions about the benefits, risks, and ethical implications of these technologies. Education and transparency are key to fostering informed consent and building trust between scientists, policymakers, and the public.
Addressing misconceptions, fears, and ethical concerns through open dialogue and public engagement can facilitate the responsible integration of bioengineering advancements into society.
Respecting diverse cultural values and ethical perspectives will also ensure that bioengineering practices are inclusive and considerate of the varied beliefs and norms that exist within different communities.
By prioritizing societal acceptance and cultural sensitivity, bioengineering can be developed and implemented in ways that are respectful, ethical, and aligned with the collective well-being of humanity.
Balancing Innovation with Sustainability
Balancing innovation with safeguards for long-term sustainability is another critical ethical challenge. While the pursuit of scientific progress drives bioengineering forward, it is essential to implement safeguards that prevent the misuse or unintended consequences of these technologies.
This involves establishing ethical guidelines that prioritize safety, environmental protection, and the well-being of future generations. Also, fostering a culture of responsibility and accountability within the scientific community ensures that bioengineering advancements are pursued with caution and foresight.
By integrating ethical considerations into every stage of research and development, from conception to implementation, bioengineering can achieve sustainable and beneficial outcomes without compromising the integrity of natural systems or societal values. The balance between fostering innovation and maintaining ethical safeguards is crucial for ensuring that bioengineering technologies contribute positively to human progress and environmental sustainability.
Bioengineering, Physical Devices, and Language Models
The advent of sophisticated physical devices and the utilization of advanced language models have further propelled bioengineering into new realms of innovation and efficiency.
This chapter delves into the intersection of bioengineering, physical devices, and language models, providing 20 comprehensive code examples that illustrate their practical applications. These examples span data analysis, device control, simulation, and natural language processing, demonstrating how these technologies synergize to address complex challenges in bioengineering.
Data Analysis in Bioengineering
Data analysis is a cornerstone of bioengineering, enabling researchers to interpret vast datasets, identify patterns, and derive meaningful insights. Python, with its extensive libraries, is a preferred language for bioinformatics and data science tasks. Below are several code examples demonstrating data analysis techniques in bioengineering.
Genomic Data Visualization
Visualizing genomic data helps in understanding genetic variations and their implications. The following example uses pandas
and matplotlib
to visualize single nucleotide polymorphisms (SNPs) across different chromosomes.
import pandas as pd
import matplotlib.pyplot as plt
# Load SNP data
snp_data = pd.read_csv('snp_data.csv') # Columns: Chromosome, Position, SNP_Type
# Plot SNP distribution per chromosome
plt.figure(figsize=(12, 6))
for chromosome in snp_data['Chromosome'].unique():
chr_data = snp_data[snp_data['Chromosome'] == chromosome]
plt.scatter(chr_data['Position'], [chromosome]*len(chr_data), label=f'Chr {chromosome}', alpha=0.6)
plt.xlabel('Position')
plt.ylabel('Chromosome')
plt.title('SNP Distribution Across Chromosomes')
plt.legend(bbox_to_anchor=(1.05, 1), loc='upper left')
plt.tight_layout()
plt.show()
This script reads SNP data from a CSV file, filters the data by each chromosome, and plots the SNP positions using scatter plots. Each chromosome is represented on the y-axis, allowing for a clear visualization of SNP distribution across the genome.
Differential Gene Expression Analysis
Identifying differentially expressed genes between conditions is crucial in understanding disease mechanisms. This example uses the statsmodels
library to perform statistical analysis on gene expression data.
import pandas as pd
import statsmodels.api as sm
# Load gene expression data
expression_data = pd.read_csv('gene_expression.csv') # Rows: Genes, Columns: Samples
conditions = pd.read_csv('conditions.csv') # Columns: Sample, Condition
# Prepare design matrix
conditions_encoded = pd.get_dummies(conditions['Condition'], drop_first=True)
X = sm.add_constant(conditions_encoded)
results = {}
# Perform t-test for each gene
for gene in expression_data['Gene']:
y = expression_data[expression_data['Gene'] == gene].iloc[0, 1:]
model = sm.OLS(y, X).fit()
p_value = model.pvalues['Condition_Treated']
results[gene] = p_value
# Convert results to DataFrame
results_df = pd.DataFrame.from_dict(results, orient='index', columns=['p_value'])
significant_genes = results_df[results_df['p_value'] < 0.05]
print(f"Number of significantly differentially expressed genes: {len(significant_genes)}")
print(significant_genes)
This script performs differential gene expression analysis by fitting an Ordinary Least Squares (OLS) model for each gene, comparing treated versus control conditions. Genes with p-values below 0.05 are considered significantly differentially expressed.
Simulation and Modeling in Bioengineering
Simulating biological systems allows for the prediction of behaviors under various conditions without the need for extensive laboratory experiments. Tools like SciPy
and NumPy
are instrumental in building and solving biological models.
Modeling Enzyme Kinetics with Michaelis-Menten Equation
The Michaelis-Menten equation describes the kinetics of enzyme-mediated reactions. This example uses SciPy
to fit experimental data to the equation.
import numpy as np
import matplotlib.pyplot as plt
from scipy.optimize import curve_fit
# Define Michaelis-Menten equation
def michaelis_menten(S, Vmax, Km):
return (Vmax * S) / (Km + S)
# Experimental data: Substrate concentration (S) and reaction rate (V)
S = np.array([0.1, 0.5, 1, 2, 5, 10, 20, 50, 100])
V = np.array([0.05, 0.2, 0.35, 0.55, 0.8, 0.95, 1.1, 1.15, 1.2])
# Fit the model to data
popt, pcov = curve_fit(michaelis_menten, S, V, bounds=(0, np.inf))
Vmax, Km = popt
print(f"Estimated Vmax: {Vmax}")
print(f"Estimated Km: {Km}")
# Plot data and fitted curve
S_fit = np.linspace(0, 100, 500)
V_fit = michaelis_menten(S_fit, Vmax, Km)
plt.scatter(S, V, label='Experimental Data')
plt.plot(S_fit, V_fit, 'r-', label='Fitted Michaelis-Menten')
plt.xlabel('Substrate Concentration (mM)')
plt.ylabel('Reaction Rate (μM/min)')
plt.title('Enzyme Kinetics: Michaelis-Menten Fit')
plt.legend()
plt.show()
This script fits experimental substrate concentration and reaction rate data to the Michaelis-Menten equation using non-linear curve fitting. The estimated parameters Vmax
and Km
provide insights into the enzyme's efficiency and affinity for the substrate.
Population Growth Modeling with Logistic Equation
The logistic growth model describes population growth with a carrying capacity. This example simulates population growth over time using the logistic equation.
import numpy as np
import matplotlib.pyplot as plt
from scipy.integrate import odeint
# Define logistic growth differential equation
def logistic_growth(P, t, r, K):
dPdt = r * P * (1 - P / K)
return dPdt
# Parameters
r = 0.3 # Growth rate
K = 1000 # Carrying capacity
P0 = 10 # Initial population
t = np.linspace(0, 30, 300) # Time
# Solve ODE
P = odeint(logistic_growth, P0, t, args=(r, K))
# Plot results
plt.plot(t, P, label='Population')
plt.axhline(y=K, color='r', linestyle='--', label='Carrying Capacity')
plt.xlabel('Time')
plt.ylabel('Population')
plt.title('Logistic Population Growth')
plt.legend()
plt.show()
This script models population growth using the logistic equation, which incorporates the carrying capacity K
to prevent indefinite growth. The solution is obtained using the odeint
function from SciPy
, and the population dynamics are visualized over time.
Control Systems in Bioengineering Devices
Control systems are vital in bioengineering devices to maintain desired states and respond to external stimuli. Python's control systems library (control
) facilitates the design and analysis of these systems.
Designing a PID Controller for a Temperature Regulation System
A Proportional-Integral-Derivative (PID) controller adjusts system inputs based on the error between desired and actual temperatures. This example designs a PID controller for a simulated temperature system.
import numpy as np
import matplotlib.pyplot as plt
import control as ctrl
# Define system parameters
K = 2.0 # Gain
tau = 5.0 # Time constant
system = ctrl.TransferFunction([K], [tau, 1])
# Define PID controller parameters
Kp = 3.0
Ki = 1.0
Kd = 0.5
controller = ctrl.TransferFunction([Kd, Kp, Ki], [1, 0])
# Closed-loop system
closed_loop = ctrl.feedback(controller*system, 1)
# Step response
t, y = ctrl.step_response(closed_loop)
# Plot response
plt.plot(t, y, label='Closed-loop Response')
plt.xlabel('Time (s)')
plt.ylabel('Temperature')
plt.title('PID Controller for Temperature Regulation')
plt.legend()
plt.grid(True)
plt.show()
This script models a temperature regulation system using a first-order transfer function. A PID controller is designed with specified proportional, integral, and derivative gains. The closed-loop system's step response illustrates the controller's effectiveness in achieving the desired temperature.
Stabilizing a Biological Oscillator
Biological oscillators, such as circadian rhythms, require stabilization to maintain consistent cycles. This example demonstrates stabilizing a simple oscillator using feedback control.
import numpy as np
import matplotlib.pyplot as plt
from scipy.integrate import odeint
# Define oscillator with feedback control
def oscillator_with_feedback(state, t, alpha, beta, gamma):
x, y = state
dxdt = alpha * (y - x)
dydt = x * (gamma - x) - y
return [dxdt, dydt]
# Parameters
alpha = 10.0
beta = 28.0
gamma = 8.0/3.0
# Initial state
state0 = [1.0, 1.0]
# Time points
t = np.linspace(0, 50, 10000)
# Integrate ODE
states = odeint(oscillator_with_feedback, state0, t, args=(alpha, beta, gamma))
# Plot trajectory
plt.plot(states[:,0], states[:,1])
plt.xlabel('X')
plt.ylabel('Y')
plt.title('Stabilized Biological Oscillator')
plt.grid(True)
plt.show()
This script simulates a stabilized biological oscillator using a modified Lorenz system with feedback control parameters. By adjusting alpha
, beta
, and gamma
, the system can achieve stable oscillatory behavior, mimicking biological rhythms.
Machine Learning in Bioengineering
Machine learning techniques are increasingly employed in bioengineering for predictive modeling, classification, and pattern recognition. Libraries like scikit-learn
and TensorFlow
facilitate the implementation of these techniques.
Predicting Protein-Protein Interactions
Predicting protein-protein interactions (PPIs) is essential for understanding cellular functions. This example uses a Support Vector Machine (SVM) to classify potential PPIs based on feature vectors.
import pandas as pd
from sklearn.model_selection import train_test_split
from sklearn.preprocessing import StandardScaler
from sklearn.svm import SVC
from sklearn.metrics import classification_report, confusion_matrix
# Load dataset
data = pd.read_csv('ppi_data.csv') # Columns: Feature1, Feature2, ..., Label
# Features and labels
X = data.drop('Label', axis=1)
y = data['Label']
# Split data
X_train, X_test, y_train, y_test = train_test_split(X, y, test_size=0.2, random_state=42)
# Feature scaling
scaler = StandardScaler()
X_train_scaled = scaler.fit_transform(X_train)
X_test_scaled = scaler.transform(X_test)
# Train SVM classifier
svm = SVC(kernel='rbf', C=1.0, gamma='scale')
svm.fit(X_train_scaled, y_train)
# Predict
y_pred = svm.predict(X_test_scaled)
# Evaluation
print(confusion_matrix(y_test, y_pred))
print(classification_report(y_test, y_pred))
This script trains an SVM classifier to predict PPIs using labeled feature data. The dataset is split into training and testing sets, standardized, and then used to train and evaluate the SVM model. The confusion matrix and classification report provide insights into the model's performance.
Deep Learning for Cell Image Classification
Classifying cell images is crucial in medical diagnostics. This example uses a Convolutional Neural Network (CNN) with TensorFlow
and Keras
to classify cell images into different categories.
import tensorflow as tf
from tensorflow.keras.models import Sequential
from tensorflow.keras.layers import Conv2D, MaxPooling2D, Flatten, Dense, Dropout
from tensorflow.keras.preprocessing.image import ImageDataGenerator
# Data preparation
train_datagen = ImageDataGenerator(rescale=1./255, horizontal_flip=True, rotation_range=20)
test_datagen = ImageDataGenerator(rescale=1./255)
train_generator = train_datagen.flow_from_directory(
'cell_images/train',
target_size=(64, 64),
batch_size=32,
class_mode='binary')
validation_generator = test_datagen.flow_from_directory(
'cell_images/validation',
target_size=(64, 64),
batch_size=32,
class_mode='binary')
# Build CNN model
model = Sequential([
Conv2D(32, (3,3), activation='relu', input_shape=(64, 64, 3)),
MaxPooling2D(pool_size=(2,2)),
Conv2D(64, (3,3), activation='relu'),
MaxPooling2D(pool_size=(2,2)),
Flatten(),
Dense(128, activation='relu'),
Dropout(0.5),
Dense(1, activation='sigmoid')
])
# Compile model
model.compile(optimizer='adam', loss='binary_crossentropy', metrics=['accuracy'])
# Train model
history = model.fit(
train_generator,
steps_per_epoch=100,
epochs=20,
validation_data=validation_generator,
validation_steps=50)
# Evaluate model
loss, accuracy = model.evaluate(validation_generator)
print(f'Validation Accuracy: {accuracy*100:.2f}%')
This script constructs a CNN for binary classification of cell images. The model includes convolutional layers for feature extraction, pooling layers for dimensionality reduction, and dense layers for classification. Data augmentation is applied to the training data to improve model generalization.
Natural Language Processing in Bioengineering
Natural Language Processing (NLP) facilitates the extraction of information from scientific literature and the automation of documentation tasks. Libraries like nltk
, spaCy
, and transformers
are commonly used in bioengineering applications.
Extracting Gene Names from Scientific Articles
Extracting gene names from text is essential for knowledge extraction and database population. This example uses spaCy
for Named Entity Recognition (NER) to identify gene names in scientific abstracts.
import spacy
# Load pre-trained spaCy model
nlp = spacy.load('en_core_web_sm')
# Sample abstract
abstract = """
The interaction between BRCA1 and RAD51 is critical for the repair of double-strand breaks in DNA.
Mutations in BRCA1 are associated with an increased risk of breast and ovarian cancers.
"""
# Process text
doc = nlp(abstract)
# Extract gene names (assuming they are tagged as ORG for this example)
gene_names = [ent.text for ent in doc.ents if ent.label_ == 'ORG']
print("Extracted Gene Names:", gene_names)
This script processes a scientific abstract to extract gene names using spaCy's NER capabilities. While spaCy's pre-trained models may not be optimized for gene names, this example demonstrates the approach. For more accurate gene name extraction, custom models or domain-specific libraries like BioBERT
can be employed.
Summarizing Biomedical Literature with Transformer Models
Summarizing biomedical literature helps researchers quickly grasp the essence of extensive research. This example uses the transformers
library to generate summaries of biomedical abstracts.
from transformers import pipeline
# Initialize summarization pipeline
summarizer = pipeline("summarization", model="facebook/bart-large-cnn")
# Sample abstract
abstract = """
The CRISPR-Cas9 system has revolutionized genome editing by allowing precise modifications to DNA sequences.
Its applications range from basic research to therapeutic interventions for genetic disorders.
Despite its potential, challenges such as off-target effects and delivery mechanisms remain.
Ongoing research focuses on improving specificity and developing efficient delivery vectors.
"""
# Generate summary
summary = summarizer(abstract, max_length=50, min_length=25, do_sample=False)
print("Summary:", summary[0]['summary_text'])
This script utilizes a pre-trained BART model to summarize a biomedical abstract. The pipeline
abstraction simplifies the process, allowing for easy integration of powerful transformer models in bioengineering workflows.
Security and Privacy in Bioengineering Data Handling
Handling sensitive bioengineering data necessitates robust security and privacy measures. This section provides code examples demonstrating encryption and secure data storage practices.
Encrypting Biomedical Data with Fernet
Encrypting biomedical data ensures that sensitive information remains confidential and protected from unauthorized access. This example uses the cryptography
library's Fernet module for symmetric encryption.
from cryptography.fernet import Fernet
import pandas as pd
# Generate encryption key
key = Fernet.generate_key()
cipher_suite = Fernet(key)
print(f"Encryption Key: {key.decode()}") # Store securely
# Load biomedical data
data = pd.read_csv('biomedical_data.csv')
# Convert DataFrame to bytes
data_bytes = data.to_csv(index=False).encode()
# Encrypt data
encrypted_data = cipher_suite.encrypt(data_bytes)
# Save encrypted data to file
with open('biomedical_data_encrypted.bin', 'wb') as file:
file.write(encrypted_data)
print("Data encrypted and saved successfully.")
This script generates a symmetric encryption key, encrypts a biomedical dataset, and saves the encrypted data to a binary file. Proper key management is crucial—the encryption key must be stored securely to allow data decryption when needed.
Secure Data Transmission with SSL/TLS
Transmitting biomedical data securely over networks is essential to prevent data breaches. This example demonstrates setting up a secure server-client communication using SSL/TLS in Python.
Server Code:
import socket
import ssl
# Create socket
server_socket = socket.socket(socket.AF_INET, socket.SOCK_STREAM)
server_socket.bind(('localhost', 8443))
server_socket.listen(5)
print("Server listening on port 8443...")
# Wrap socket with SSL
context = ssl.create_default_context(ssl.Purpose.CLIENT_AUTH)
context.load_cert_chain(certfile='server.crt', keyfile='server.key')
while True:
client_socket, addr = server_socket.accept()
conn = context.wrap_socket(client_socket, server_side=True)
print(f"Connection from {addr}")
data = conn.recv(1024)
if data:
print(f"Received: {data.decode()}")
conn.sendall(b"Data received securely.")
conn.close()
Client Code:
import socket
import ssl
# Create socket
client_socket = socket.socket(socket.AF_INET, socket.SOCK_STREAM)
# Wrap socket with SSL
context = ssl.create_default_context()
context.check_hostname = False
context.verify_mode = ssl.CERT_NONE # For testing purposes only
conn = context.wrap_socket(client_socket, server_hostname='localhost')
# Connect to server
conn.connect(('localhost', 8443))
print("Connected to server.")
# Send data
message = "Sensitive biomedical data."
conn.sendall(message.encode())
# Receive response
response = conn.recv(1024)
print(f"Server Response: {response.decode()}")
conn.close()
This setup establishes a secure server-client communication channel using SSL/TLS. The server listens for incoming connections, wraps the socket with SSL using a certificate and key, and securely receives data from clients. The client connects to the server using an SSL context, sends sensitive data, and receives confirmation. Note: In production, proper certificate verification should be enforced to ensure security.
Leveraging Cloud Computing for Bioengineering Applications
Cloud computing provides scalable resources for bioengineering applications, facilitating large-scale data processing and storage. This section includes examples of using cloud services for bioengineering tasks.
Uploading and Processing Data on AWS S3 with Boto3
Amazon Web Services (AWS) S3 offers scalable storage for bioengineering data. This example demonstrates how to upload a file to S3 and process it using AWS Lambda.
import boto3
from botocore.exceptions import NoCredentialsError
# Initialize S3 client
s3 = boto3.client('s3')
def upload_to_s3(file_name, bucket, object_name=None):
if object_name is None:
object_name = file_name
try:
s3.upload_file(file_name, bucket, object_name)
print(f"Uploaded {file_name} to {bucket}/{object_name}")
except FileNotFoundError:
print("The file was not found")
except NoCredentialsError:
print("Credentials not available")
# Upload file
upload_to_s3('biomedical_data.csv', 'my-bioengineering-bucket', 'data/biomedical_data.csv')
# Lambda function code (to be deployed on AWS Lambda)
"""
import json
import boto3
import pandas as pd
def lambda_handler(event, context):
s3 = boto3.client('s3')
bucket = event['Records'][0]['s3']['bucket']['name']
key = event['Records'][0]['s3']['object']['key']
# Download the file from S3
s3.download_file(bucket, key, '/tmp/biomedical_data.csv')
# Process data (example: compute mean of a column)
data = pd.read_csv('/tmp/biomedical_data.csv')
mean_values = data.mean().to_dict()
# Log results
print("Mean Values:", mean_values)
return {
'statusCode': 200,
'body': json.dumps('Data processed successfully!')
}
"""
This script uploads a biomedical data file to an AWS S3 bucket using the boto3
library. The accompanying AWS Lambda function (commented out) is triggered upon file upload, downloads the file, processes it (for example, computing mean values), and logs the results. This integration automates data processing workflows, leveraging cloud scalability and serverless computing.
Deploying a Bioinformatics Web Application on Heroku
Deploying bioinformatics tools as web applications enables broader accessibility and collaboration. This example outlines deploying a simple Flask-based bioinformatics tool on Heroku.
Flask Application (app.py
):
from flask import Flask, request, jsonify
import pandas as pd
import io
app = Flask(__name__)
@app.route('/upload', methods=['POST'])
def upload_file():
if 'file' not in request.files:
return jsonify({'error': 'No file provided'}), 400
file = request.files['file']
if file.filename == '':
return jsonify({'error': 'No selected file'}), 400
if file:
stream = io.StringIO(file.stream.read().decode("UTF8"), newline=None)
df = pd.read_csv(stream)
summary = df.describe().to_dict()
return jsonify({'summary': summary}), 200
if __name__ == '__main__':
app.run(debug=True)
Requirements (requirements.txt
):
Flask
pandas
gunicorn
Procfile:
web: gunicorn app:app
Deployment Steps:
Initialize a Git repository and commit the code.
Create a Heroku app:
heroku create bioinformatics-app
Deploy to Heroku:
git push heroku master
Access the deployed app via the provided Heroku URL.
This Flask application provides an endpoint to upload a CSV file containing bioinformatics data. Upon receiving the file, it processes the data using pandas
, generates statistical summaries, and returns the results as a JSON response. Deploying this application on Heroku makes it accessible to researchers worldwide, facilitating data analysis and collaboration.
Ethical Considerations in Bioengineering Technology Integration
Integrating advanced technologies in bioengineering raises ethical considerations related to privacy, consent, and the potential for misuse. This section emphasizes the importance of ethical practices and provides guidelines for responsible technology integration.
Ensuring Data Privacy and Security
Protecting sensitive biomedical data is paramount. Implementing robust encryption, access controls, and compliance with regulations like HIPAA ensures data privacy and security.
# Example: Implementing role-based access control (RBAC) in a Flask application
from flask import Flask, request, jsonify
from functools import wraps
app = Flask(__name__)
# Simple user roles
users = {
'admin': {'password': 'adminpass', 'role': 'admin'},
'user1': {'password': 'user1pass', 'role': 'user'}
}
def check_auth(username, password):
if username in users and users[username]['password'] == password:
return users[username]['role']
return None
def requires_role(role):
def decorator(f):
@wraps(f)
def decorated(*args, **kwargs):
auth = request.authorization
if not auth or not check_auth(auth.username, auth.password):
return jsonify({'message': 'Authentication required'}), 401
user_role = check_auth(auth.username, auth.password)
if user_role != role and user_role != 'admin':
return jsonify({'message': 'Permission denied'}), 403
return f(*args, **kwargs)
return decorated
return decorator
@app.route('/admin/data', methods=['GET'])
@requires_role('admin')
def admin_data():
# Return sensitive data
return jsonify({'data': 'Sensitive admin data'}), 200
@app.route('/user/data', methods=['GET'])
@requires_role('user')
def user_data():
# Return user-specific data
return jsonify({'data': 'User-specific data'}), 200
if __name__ == '__main__':
app.run(debug=True)
This Flask application implements basic role-based access control (RBAC) to restrict access to sensitive data based on user roles. Only users with the 'admin' role can access admin-specific data, while regular users can access their own data.
Enhancing such mechanisms with more sophisticated authentication and authorization frameworks ensures robust data privacy and security.
Addressing Bias in Machine Learning Models
Machine learning models can inadvertently perpetuate biases present in training data. Ensuring diverse and representative datasets and implementing bias detection mechanisms are essential for fair and ethical AI applications in bioengineering.
from sklearn.model_selection import train_test_split
from sklearn.ensemble import RandomForestClassifier
from sklearn.metrics import classification_report
import pandas as pd
# Load dataset with demographic information
data = pd.read_csv('patient_data.csv') # Includes features and 'Outcome'
# Check for bias
print(data['Demographic'].value_counts())
# Ensure balanced dataset
X = data.drop(['Outcome', 'Demographic'], axis=1)
y = data['Outcome']
demographics = data['Demographic']
X_train, X_test, y_train, y_test, dem_train, dem_test = train_test_split(
X, y, demographics, test_size=0.2, stratify=demographics, random_state=42)
# Train model
clf = RandomForestClassifier(n_estimators=100, random_state=42)
clf.fit(X_train, y_train)
# Predict
y_pred = clf.predict(X_test)
# Evaluate
print(classification_report(y_test, y_pred))
# Analyze performance across demographics
for group in dem_test.unique():
idx = dem_test == group
print(f"Performance for {group}:")
print(classification_report(y_test[idx], y_pred[idx]))
This script evaluates the performance of a Random Forest classifier across different demographic groups to detect potential biases. By stratifying the dataset and analyzing classification metrics for each group, bioengineers can identify and mitigate biases, ensuring equitable AI-driven decisions in healthcare applications.
Future Directions in Bioengineering Technology Integration
The integration of bioengineering with physical devices and language models is poised to drive significant advancements in healthcare, biotechnology, and environmental sustainability. Future directions include the development of intelligent biomedical devices, enhanced data interpretation systems, and collaborative platforms that leverage the strengths of each technology.
Intelligent Wearables for Personalized Health Monitoring
Wearable devices embedded with intelligent algorithms and language models can provide personalized health insights and proactive healthcare management. Future developments may include continuous monitoring of vital signs, real-time health analytics, and seamless communication with healthcare providers through natural language interfaces.
# Example: Integrating wearable sensor data with a chatbot for health advice
import speech_recognition as sr
from transformers import pipeline
# Initialize speech recognizer and summarization pipeline
recognizer = sr.Recognizer()
command_processor = pipeline("text2text-generation", model="t5-small")
def listen_for_commands():
with sr.Microphone() as source:
print("Listening for commands...")
audio = recognizer.listen(source)
try:
command = recognizer.recognize_google(audio)
print(f"Recognized Command: {command}")
return command
except sr.UnknownValueError:
print("Could not understand audio")
return ""
except sr.RequestError as e:
print(f"Could not request results; {e}")
return ""
def process_command(command):
if "open hand" in command.lower():
action = "Opening hand"
elif "close hand" in command.lower():
action = "Closing hand"
else:
action = "Command not recognized"
return action
def main():
while True:
command = listen_for_commands()
if command:
action = process_command(command)
print(action)
# Here, integrate with prosthetic control system
# e.g., send_signal_to_prosthetic(action)
if __name__ == "__main__":
main()
This script captures verbal commands using a microphone, processes them with a speech recognizer, and interprets the commands using simple keyword matching. In a real-world scenario, the process_command
function could be enhanced with a language model to better understand and execute complex instructions, directly interfacing with the prosthetic's control system to perform actions like opening or closing the hand.
Collaborative Platforms for Bioengineering Research
Collaborative platforms that integrate bioengineering data, physical devices, and language models can facilitate interdisciplinary research and innovation. These platforms can support data sharing, joint analysis, and automated reporting, fostering a more integrated and efficient research environment.
# Example: Building a collaborative dashboard with Streamlit
import streamlit as st
import pandas as pd
from transformers import pipeline
# Initialize summarization pipeline
summarizer = pipeline("summarization", model="facebook/bart-large-cnn")
st.title("Bioengineering Collaborative Dashboard")
# File upload
uploaded_file = st.file_uploader("Upload Bioengineering Data (CSV)", type="csv")
if uploaded_file:
data = pd.read_csv(uploaded_file)
st.write("Data Preview:")
st.dataframe(data.head())
# Data summary
if st.button("Generate Summary"):
summary = data.describe().to_string()
st.write("Data Summary:")
st.text(summary)
# Summarize summary using language model
lm_summary = summarizer(summary, max_length=100, min_length=50, do_sample=False)[0]['summary_text']
st.write("Automated Summary:")
st.write(lm_summary)
This Streamlit application allows researchers to upload bioengineering datasets, view data previews, generate statistical summaries, and obtain automated summaries using a language model. Such collaborative dashboards enhance data accessibility and streamline research workflows.
The integration of bioengineering with physical devices and language models represents a transformative frontier in scientific and medical advancements. By harnessing the capabilities of data analysis, simulation, machine learning, and natural language processing, bioengineers can develop intelligent systems that enhance healthcare outcomes, drive biotechnological innovations, and address environmental challenges.
Ethical considerations, security measures, and equitable access remain paramount in ensuring that these technologies are deployed responsibly and beneficially. As we move forward, the collaborative efforts of bioengineers, data scientists, and AI specialists will be crucial in shaping a future where technology and biology synergize to create a healthier and more sustainable world.
How to Get Started in Bioengineering
Bioengineering is a multifaceted field that combines biology, engineering, and technology to solve real-world problems, and entering this domain requires a blend of passion, perseverance, and practical planning.
To start, it is essential to understand what bioengineering entails: it involves designing innovative solutions—from 3D bioprinting organs to engineering crops for climate resilience—and why these advancements matter for society. Aspiring bioengineers should ask themselves how their interests in science and technology can contribute to groundbreaking research and tangible healthcare or environmental outcomes.
It’s crucial to build a strong academic foundation in core subjects such as biology, chemistry, physics, and mathematics, as these disciplines provide the technical bedrock for advanced bioengineering concepts.
Early on, students must identify which aspect of bioengineering ignites their passion by exploring various subfields through online courses, lectures, or lab visits, and by asking why they want to make an impact and how they can use technology to solve pressing global challenges.
Diverse Pathways to Entry: Formal Education and Self-Directed Learning
Pursuing formal education is a critical step, but in today’s interconnected world, university is not the only entry point into bioengineering.
While accredited programs in bioengineering, biomedical engineering, or biotechnology provide invaluable structure and research opportunities, self-directed learning has never been more accessible. Open-source software, free online courses, and community-driven platforms can help you access cutting-edge applications and contribute to innovative projects—all from the comfort of your own garage or home lab.
If you’re a prospective bioengineer, you should explore digital communities on GitHub, Stack Overflow, or dedicated bioengineering forums to collaborate on projects, share ideas, and even contribute to open-source bioinformatics tools.
Practical details matter: inquire about research opportunities, internships, and industry partnerships, and ask when these opportunities typically become available during the program, or even how you can replicate these experiences independently.
By combining formal education with independent exploration, you create a hybrid model of learning that is both flexible and powerfully effective in today’s technological landscape.
Gaining Practical Experience: Bridging Theory and Hands-On Innovation
Beyond the classroom or self-taught endeavors, developing practical experience and soft skills is imperative to transform your academic knowledge into industry-ready expertise.
Seek out internships, research assistantships, or independent projects early in your journey to learn what a day in the life of a bioengineer looks like, and ask yourself what specific challenges you are most excited to tackle. These experiences, whether obtained in a structured corporate or academic setting or through hands-on projects in your garage, provide the opportunity to work with cutting-edge technology and learn how interdisciplinary teams solve complex problems collaboratively.
When seeking internships or personal projects, focus on opportunities that emphasize innovation and mentorship, ensuring that you receive feedback and guidance from experienced professionals.
Networking is another critical component. Try to attend industry conferences, seminars, and local meet-ups or join online communities where you can connect with thought leaders, potential mentors, and peers who share your passion.
This proactive approach will help you develop the communication, leadership, and project management skills indispensable in any engineering discipline.
Balancing Innovation with Ethics: Responsible Research in Bioengineering
A strategic career in bioengineering demands a careful balance between innovation and ethical responsibility, ensuring that your contributions benefit society without unintended negative consequences.
Make sure you understand why ethical considerations are paramount in this field, as you are dealing with technologies that can fundamentally alter life and impact ecosystems. Ask yourself how you can implement best practices for ethical research, such as obtaining proper consent for clinical trials, ensuring data privacy, and minimizing environmental impacts when engineering organisms.
Practical steps include enrolling in ethics courses, attending workshops on regulatory frameworks, and staying informed about guidelines set by professional organizations such as the Biomedical Engineering Society or the World Health Organization.
It is vital to know when to pause and reflect on the potential societal implications of your work, whether you are designing a new medical device or developing genetically modified crops. By integrating ethical practices into every stage of research and development, you build a reputation for responsible innovation—a quality highly valued by employers, investors, and regulatory bodies alike.
Mapping Your Career: Setting Goals and Achieving Milestones
Creating a practical and actionable roadmap for your bioengineering career is the final, yet most crucial, component of your strategic blueprint. Begin by setting clear, time-bound goals that outline what you aim to achieve in the short, medium, and long term—whether it is securing an internship, publishing a research paper, or leading an independent project that began in your garage.
Ask yourself when each milestone should be reached, and why these benchmarks are critical for your professional growth. Develop a detailed plan that includes acquiring specialized skills through workshops, online courses, or certifications in areas such as computational biology, synthetic biology, and bioinformatics.
You should also keep a detailed portfolio of your projects, maintain an updated résumé, and actively seek mentorship. All this will help provide the guidance you need to navigate the competitive job market.
Use digital tools and career planning software to track your progress, set reminders for application deadlines, and schedule networking events. Understanding how to balance academic pursuits with industry exposure—and why continuous learning is vital—will empower you to adjust your strategy in response to emerging trends.
By following your comprehensive roadmap, you can transform your aspirations into tangible achievements and secure a rewarding career in the dynamic world of bioengineering.
Conclusion: Responsible Stewardship of Bioengineering
The future of bioengineering is poised to revolutionize various aspects of human life, from healthcare and longevity to environmental sustainability and technological innovation. But the realization of this potential hinges on our ability to navigate the ethical, regulatory, and societal challenges that accompany these advancements.
By fostering responsible stewardship, promoting equitable access, and engaging in inclusive dialogue, we can ensure that bioengineering serves the greater good and contributes to a sustainable and thriving future for all.
The integration of diverse perspectives and the commitment to ethical principles will be paramount in shaping the trajectory of bioengineering, ensuring that its benefits are harnessed responsibly and effectively.
As we stand on the cusp of unprecedented scientific breakthroughs, the collaborative efforts of scientists, policymakers, and communities will determine the legacy of bioengineering in shaping the future of humanity and our planet.
Transform Your Future with Data Science & AI
Ready to break into the booming field of Data Science and AI? Download our free eBook, Six-Figure Data Science Bootcamp, and discover the exact steps to build in-demand skills, gain real-world experience, and land your dream job.